Metabolic flexibility
Metabolic flexibility is the ability of an organism to adapt efficiently and rapidly to different metabolic and energy states.
Healthy young individuals are able to transition easily between feeding states (fed versus fasting) and between different fuels (carbohydrates versus fats) as sources of energy. On the contrary, obesity and aging appear to share in common the inability to maintain metabolic flexibility.[1]
The general public is becoming more aware of the importance of metabolic flexibility, and companies like Lumen Metabolism and Levels are currently offering personalized dietary recommendations based on the measurement of an individuals’s metabolic flexibility.
Metabolic slowdown
Metabolic slowdown is consistently observed with ageing. Starting around age 60, resting metabolic rates (RMRs) declines by approximately 1% annually.[2][3] In humans basal metabolic rate declines with age independent of the decline in fat-free mass (FFM) and a physically active lifestyle can only partly protect against loss of FFM in aging adults.[4][5] It is also evident in mice,[6][7] cats and dogs.[8]
AMPK and mTORC1 as central energy and nutrient sensors
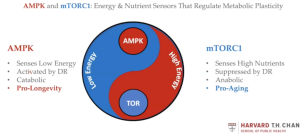
There are a variety of sensors controlling metabolic flexibility in an organism. The best known systems for metabolic flexibility are AMPK and mTORC1, both highly conserved across species including nematodes, flies, mice and humans.
AMPK (AMP-activated protein kinase), is activated under catabolic conditions when intracellular ATP production is decreased. AMPK has key roles in regulating cellular growth, metabolism and autophagy. It is known as a pro-longevity pathway and is activated by physiological states such as fasting or exercise.
On the other hand, the mTOR pathway (and specifically mTORC1) acts as the anabolic counterpart and functions to sense fed states or high availability of nutrients. It is a known pro-aging pathway and is suppressed by metabolic states like fasting or caloric restriction. Interventions such as rapamycin inhibit mTORC1 and are therefore believed to have a pro-longevity effect. Ubiquitous inhibition of mTORC1 extends lifespan in multiple organisms but also disrupts several anabolic processes resulting in stunted growth, slowed development, reduced fertility, and disrupted metabolism. It has been shown that targeting mTORC1 specifically in the nervous system in C. elegans (for example, using neuron-specific degradation of RAGA-1, an upstream activator of mTORC1) uncouples longevity from growth and reproductive impairments, and thus many canonical effects of low mTORC1 activity are not required to promote healthy aging.[11]
Mediators of metabolic flexibility
The research group led by William Mair’s lab at Harvard University, proposes that loss of this metabolic flexibility during aging (ie. the proper activation/suppression of mTOR/AMPK pathways) is the main risk factor for the onset of age-related diseases.[12] Following this hypothesis, Mair’s lab is focused on defining molecular mediators of distinct metabolic states to develop novel therapeutic strategies against age-related diseases.
An example of such mediators in C. elegans are CRTCs (CREB-regulated transcriptional coactivators) and the transcription factor CREB (cAMP-response element binding protein).[13] CRTCs are a family of cofactors involved in a variety of physiological processes such as energy homeostasis in insulin-sensitive tissues.[14] They are conserved in humans and have been linked to age-related diseases such as type-2 diabetes.[15]
Constitutive activation of AMPK can be achieved by disrupting an energy sensor component of the AMPK complex and leads to a lifespan extension of 50% in C. elegans.[13] This intervention genetically mimics dietary restriction despite worms being fed ad libitum. Activation of AMPK and inhibition of calcineurin extend lifespan via CRTCs and CREB in the nervous tissue and requires remodelling of the mitochondria and peroxisome network.[13] More recently, it has been shown that CRTC-1 in the neurons drives cell non-autonomous regulation of mitochondrial dynamics in other tissues and modulate lifespan.[16]
Mitochondrial dynamics
Mitochondria are highly dynamic organelles found in most eukaryotes and commonly referred to as the powerhouses of the cell. Imaging studies in live cells show that mitochondria undergo coordinated cycles of fission and fusion in order to maintain their shape, distribution and size.[17][18]
The activity of mitochondrial dynamics is highly context specific; its cycles of fission and fusion will depend on age of the animal, nutrient conditions (fasting versus feeding) and whether there is cellular damage. Besides metabolic flexibility, mitochondria dynamics also facilitate mitophagy, the autophagy of mitochondria. Mitochondrial dysfunction is a hallmark of aging and has been implicated in age-related diseases such as Alzheimer’s and Parkinson’s disease.[19]
A key process required for efficient metabolic flexibility is the remodelling of the mitochondrial network.[12] In other words, cells require mitochondrial dynamics to respond to certain changes in nutrient availability, cellular stresses and other molecular signals. For instance, during fasting mitochondria fuse together to enable more efficient respiration or metabolism, whilst during feeding states, mitochondria fragment, facilitating their mitophagy.
Mitochondrial dynamics in aging
During aging, wild-type worms fed ad libitum display an increasingly disorganised and unstructured network of mitochondria. Intermitting fasting remodels the mitochondrial network with a more structured state during fasting states and a more disorganised morphology during fed states.[12]
The lifespan extension observed during both AMPK activation and calorie restriction (CR) requires mitochondrial network remodelling in C. elegans.[12] Animals with adynamic, permanently fused mitochondria are unable to respond to intermittent fasting (IF) and no longer have the associated lifespan extension.[12] This means IF requires functional mitochondrial dynamics for its associated lifepan increase.
Surprisingly, permanently fusing mitochondria during aging to prevent fission and fusion is sufficient to double maximum lifespan in worms.[12] This is believed to be due to more stable mitochondrial networks, which can no longer become unstructured during aging. However, these worms are no longer able to respond to metabolic stresses and therefore do not have additional lifespan extension from AMPK activation or calorie restriction interventions.
Downstream of mitochondrial network homeostasis, peroxisome function and fatty acid oxidation are required to promote longevity during conditions of CR and AMPK activation.[12]
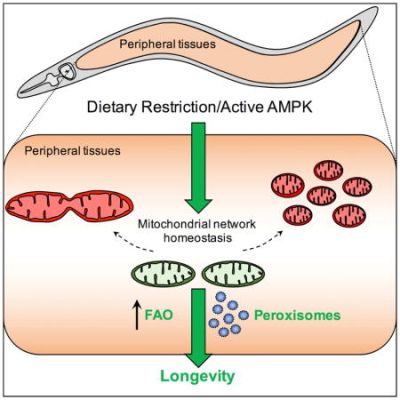
References
- ↑ Smith, R., Soeters, M., Wüst, R., & Houtkooper, R. (2018). Metabolic Flexibility as an Adaptation to Energy Resources and Requirements in Health and Disease. Endocrine Reviews, 39(4), 489-517. doi: 10.1210/er.2017-00211
- ↑ Pontzer, H., Yamada, Y., Sagayama, H., Ainslie, P. N., Andersen, L. F., Anderson, L. J., ... & IAEA DLW Database Consortium §. (2021). Daily energy expenditure through the human life course. Science, 373(6556), 808-812. PMID: 34385400 PMC8370708 DOI: 10.1126/science.abe5017
- ↑ Willis, E. A., Herrmann, S. D., Hastert, M., Kracht, C. L., Barreira, T. V., Schuna Jr, J. M., ... & Ainsworth, B. E. (2024). Older Adult Compendium of Physical Activities: Energy costs of human activities in adults aged 60 and older. Journal of Sport and Health Science, 13(1), 13-17. PMID: 38242593 PMC10818108 DOI: 10.1016/j.jshs.2023.10.007
- ↑ Westerterp, K. R., Yamada, Y., Sagayama, H., Ainslie, P. N., Andersen, L. F., Anderson, L. J., ... & Speakman, J. R. (2021). Physical activity and fat-free mass during growth and in later life. The American journal of clinical nutrition, 114(5), 1583-1589. PMID: 34477824 PMC8574623 DOI: 10.1093/ajcn/nqab260
- ↑ Heymsfield, S. B., & Fearnbach, N. (2021). Can increasing physical activity prevent aging-related loss of skeletal muscle?. The American Journal of Clinical Nutrition, 114(5), 1579-1580. PMID: 34476475 PMC8574632 DOI: 10.1093/ajcn/nqab283
- ↑ Houtkooper, R. H., Argmann, C., Houten, S. M., Cantó, C., Jeninga, E. H., Andreux, P. A., ... & Auwerx, J. (2011). The metabolic footprint of aging in mice. Scientific reports, 1(1), 134.
- ↑ Rogers, N. H., Landa, A., Park, S., & Smith, R. G. (2012). Aging leads to a programmed loss of brown adipocytes in murine subcutaneous white adipose tissue. Aging cell, 11(6), 1074-1083. PMID: 23020201 PMC3839316 DOI: 10.1111/acel.12010
- ↑ Groves, E. (2019). Nutrition in senior cats and dogs: how does the diet need to change, when and why?. Companion Animal, 24(2), 91-101.
- ↑ Xu, J., Ji, J., & Yan, X. (2012). Cross-Talk between AMPK and mTOR in Regulating Energy Balance. Critical Reviews In Food Science And Nutrition, 52(5), 373-381. doi: 10.1080/10408398.2010.500245
- ↑ https:://vimeo.com/523565896
- ↑ Smith HJ, Lanjuin A, Sharma A, Prabhakar A, Nowak E, Stine PG, et al. (2023) Neuronal mTORC1 inhibition promotes longevity without suppressing anabolic growth and reproduction in C. elegans. PLoS Genet 19(9): e1010938. https://doi.org/10.1371/journal.pgen.1010938
- ↑ Jump up to: 12.0 12.1 12.2 12.3 12.4 12.5 12.6 Weir, H., Yao, P., Huynh, F., Escoubas, C., Goncalves, R., & Burkewitz, K. et al. (2017). Dietary Restriction and AMPK Increase Lifespan via Mitochondrial Network and Peroxisome Remodeling. Cell Metabolism, 26(6), 884-896.e5. doi: 10.1016/j.cmet.2017.09.024
- ↑ Jump up to: 13.0 13.1 13.2 Mair, W., Morantte, I., Rodrigues, A., Manning, G., Montminy, M., Shaw, R., & Dillin, A. (2011). Lifespan extension induced by AMPK and calcineurin is mediated by CRTC-1 and CREB. Nature, 470(7334), 404-408. doi: 10.1038/nature09706
- ↑ Altarejos, J., & Montminy, M. (2011). CREB and the CRTC co-activators: sensors for hormonal and metabolic signals. Nature Reviews Molecular Cell Biology, 12(3), 141-151. doi: 10.1038/nrm3072
- ↑ Berdeaux, R., & Hutchins, C. (2019). Anabolic and Pro-metabolic Functions of CREB-CRTC in Skeletal Muscle: Advantages and Obstacles for Type 2 Diabetes and Cancer Cachexia. Frontiers In Endocrinology, 10. doi: 10.3389/fendo.2019.00535
- ↑ Zhang, Y., Lanjuin, A., Chowdhury, S., Mistry, M., Silva-García, C., & Weir, H. et al. (2019). Neuronal TORC1 modulates longevity via AMPK and cell nonautonomous regulation of mitochondrial dynamics in C. elegans. Elife, 8. doi: 10.7554/elife.49158
- ↑ Okamoto, K., & Shaw, J. (2005). Mitochondrial Morphology and Dynamics in Yeast and Multicellular Eukaryotes. Annual Review Of Genetics, 39(1), 503-536. doi: 10.1146/annurev.genet.38.072902.093019
- ↑ Tilokani, L., Nagashima, S., Paupe, V., & Prudent, J. (2018). Mitochondrial dynamics: overview of molecular mechanisms. Essays In Biochemistry, 62(3), 341-360. doi: 10.1042/ebc20170104
- ↑ Bonda DJ, Smith MA, Perry G, Lee HG, Wang X, Zhu X. The mitochondrial dynamics of Alzheimer’s disease and Parkinson’s disease offer important opportunities for therapeutic intervention. Curr Pharm Des. 2011;17:3374–3380.
- ↑ Weir, H., Yao, P., Huynh, F., Escoubas, C., Goncalves, R., & Burkewitz, K. et al. (2017). Dietary Restriction and AMPK Increase Lifespan via Mitochondrial Network and Peroxisome Remodeling. Cell Metabolism, 26(6), 884-896.e5. doi: 10.1016/j.cmet.2017.09.024