Aging and neurodegeneration
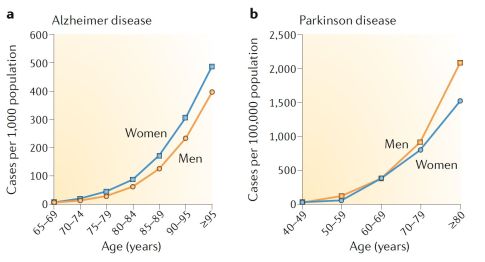
Aging is the major risk factor for most neurodegenerative diseases, such as Alzheimer's disease and Parkinson's disease.[2] The most common types of neurodegenerative diseases primarily occur in older individuals. In fact, 1 in 10 individuals over 65 years old is expected to suffer a neurodegenerative condition, with that likelihood increasing exponentially with age.[2]
Neurodegenerative diseases of the central nervous system (CNS) are defined by the progressive and irreversible loss of neuronal cells and is associated to behavioral impairment, including loss of motor and/or cognitive functions.[3]
Given the strong link between neurodegenerative diseases and aging, neurodegeneration is often considered as part of the aging process in the brain.[2]
Very few or no effective treatments are available for neurodegenerative conditions, and most are focused on alleviating the symptoms rather than targeting the root of the disease. Therefore, addressing the brain's aging process directly to slow its progression may offer a better strategy to mitigate the onset and burden of neurodegenerative diseases.
Risk factors for neurodegenerative diseases
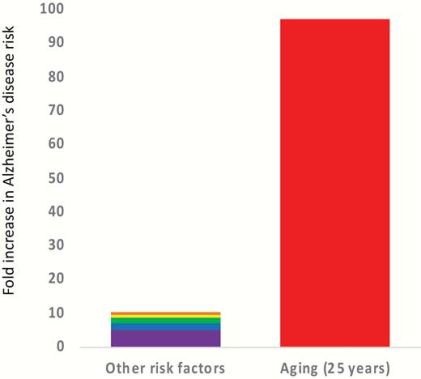
There are a number of risk factors for neurodegenerative diseases.
Aging
Aging is the most significant risk factor for several neurodegenerative diseases. Over the age of 65, over 10% of individuals are reported to have Alzheimer's disease, and the prevalence continues to increase exponentially with age. By the age of 95, over 50% of individuals are estimated to have Alzheimer's disease in the USA.[5]
The increased risk of Alzheimer's disease due to aging is over 100-fold, whereas other major risk factors (genetics, environmental and developmental factors) combined are approximately 10-fold. [4]
Genetic risk factors
In most common neurodegenerative diseases, there are known genetic risk factors that explain to varying degrees the onset and advance of neurodegeneration. Some neurodegenerative diseases are monogenic, meaning that they are caused by a single gene, as is the case of Huntington's disease (which is caused by an abnormal repeat expansion of the Huntingtin HTT gene). However, most neurodegenerative diseases including Alzheimer's disease, Amyotrophic Lateral Sclerosis (ALS) or Parkinson's disease, display a complex genetic relationship and are associated with a plethora of genetic markers, each contributing a small effect size to the disease phenotype.[6]
For Alzheimer's disease and Parkinson's disease, genetic variants with high penetrance have identified β-amyloid and α-synuclein proteins that lead to pathology, respectively. Variants with high penetrance often lead to familial forms of the disease, which are characterized for early onset and low prevalence in the population, accounting for less than 1% of cases in Alzheimer's disease. [7] Familial cases have nonetheless been subject to considerable research interest, hoping to identify common disease mechanisms.[8]
In sporadic forms of the disease (occurring with no apparent pattern), Genome-Wide Association Studies (GWAS) have been able to identify important risk-alleles, such as mutations in the APOE gene for Alzheimer's disease, or mutations in the SNCA and MAPT genes for Parkinson's. Mutations associated with Alzheimer's disease and Parkinson's disease do not seem to overlap to a significant degree.[9]
Polymorphisms in the APOE gene, a gene which encodes a protein mainly involved in lipid metabolism, is the best-described genetic factor for Alzheimer's disease. APOE has three major variants, ε2, ε3 and ε4. Whilst the ε2 allele confers a reduced risk for Alzheimer's disease (0.56-fold risk for heterozygous and homozygous carriers) compared to the most common ε3 allele, ε4 has shown to be a major risk factor for Alzheimer's disease (3.63-fold risk for heterozygous carriers and 14.49-fold for homozygous carriers).[10]
Environmental toxins
Long-term exposure to environmental toxins has been described as a risk factor for various neurodegenerative diseases.[11] Environmental toxins most commonly refers to metals toxins (such as zinc, copper or mercury) and biotoxins, which are toxic substances of a biological origin (bacteria, plants or fungi).
Environmental toxins have been shown to play a role in inducing oxidative stress and up-regulating pro-inflammatory cytokines, which can result in increased inflammatory responses and neuronal damage.[11] Some studies have found that metal toxins and biotoxins interact together in a complex manner, and that the nature of this relationship can determine the degree of impairment in neurological function.[12]
Chronic exposure to environmental toxins has been directly implicated in amyloid-β aggregation and τ-hyperphosphorylation, two pathological traits that lead to the formation of amyloid plaques and neurofibrillary tangles characteristic of Alzheimer's disease.[11][13]. Moreover, fetal and even epigenetic mechanisms (before pregnancy), due to maternal exposure to such toxins has been proposed to be the underlying cause of the phenotypic diversity and likelihood of developing neurodegenerative diseases.[14]
How to reduce the risk of exposure to environmental toxins?
Human activity has led to increased levels of environmental toxins that can be harmful to humans and other species. Whilst avoiding heavy metals and other toxins in our daily lives might prove to be challenging, health experts have proposed a number of habits to reduce the risk of exposure to environmental toxins.[15]
These habits include but are not limited to:
- Avoiding the use of pesticides whenever possible. This includes direct exposure to pesticides as well as the preferential consumption of organic food, given that chemical-synthetic pesticides are banned in organic farming.
- Handling wild mushrooms properly before consumption. The outer peel of mushrooms, especially those grown near industrial areas, has been shown to accumulate high levels of heavy metals.[16]
- Avoiding products contained in aluminium cans (such as beverages, deodorants and other cosmetic products).
- Eating seafood with moderation. Despite the fact that consumption of fish and seafood is associated to a reduced risk of cardiovascular disease, a moderate consumption is advised. High mercury levels are observed amongst high-end fish and seafood consumers and it can be particularly dangerous for pregnant women.[17]
Head trauma
Head trauma is a risk factor for a variety of neurodegenerative diseases, such as Alzheimer's disease, Parkinson's disease, Amyotrophic Lateral Sclerosis (ALS), FrontoTemporal Dementia (FTD) and chronic traumatic encephalopathy and raises the risk of all-cause dementia by a factor of approximately 1.5.[18] Head trauma has been associated with defects in the blood-brain barrier, neuroinflammation, τ-hyperphosphorylation and TDP-43 aggregation.[19]
Social environmental factors
Social environmental conditions during childhood have been suggested as a risk factor for neurodegenerative diseases. Lower socioeconomic status during early life and childhood trauma appears to be associated with decreased late-life cognitive abilities, but does not directly influence the risk of Alzheimer's disease or the rate of cognitive decline.[20]
Lower education levels and lower general cognitive abilities in childhood are associated to a higher risk of developing Alzheimer's disease and to a lower cognitive reserve .[21][22] Cognitive reserve (CR) is defined as the resilience that individuals display in terms of behavioral function when there is existing brain damage. Individuals with higher CR will showcase a lesser degree of impairment than individuals with lower CR, when both are subject to the same degree of observable brain damage. In other words, individuals with a higher cognitive reserve are likely to remain functional for longer. Higher levels of education and cognitively complex occupations are associated to a higher CR and seem to be protective against the onset of Alzheimer's disease.[23]
Brain aging and functional decline
The brain tissue of older adults is associated to the build-up of protein deposits such as tau protein and amyloid-β.
The Hallmarks of Aging
See the full article on the Hallmarks of Aging.
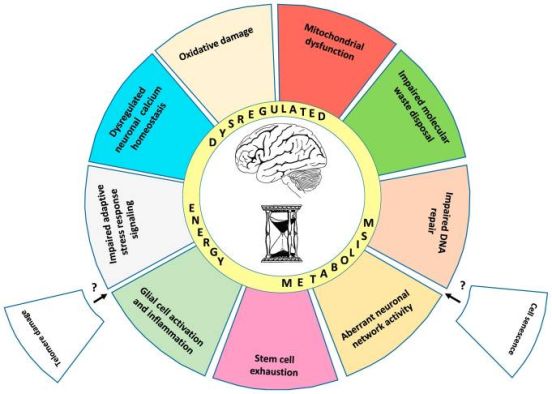
The basic process of neurodegeneration appears to be fundamentally connected to the hallmarks of aging.
The nine so-called "Hallmarks of Aging" were defined in 2013 by Lopez-Otin et al.[25] and represent the basic biological processes underlying aging at the organismal level. These hallmarks are now widely used in the aging field and in the context of neurodegenerative diseases.
The hallmarks are: genomic instability, epigenetic alterations, telomere attrition, loss of proteostasis, mitochondrial dysfunction, cellular senescence, deregulated nutrient sensing, stem cell exhaustion and altered intercellular communication. These hallmarks have shown to correlate with risk to neurodegenerative diseases.[2]
Importantly, these hallmarks offer a phenotypic observation of features associated with aging, but might fail to provide a definitive causal explanation to the aging process (see Critical Review).
The Hallmarks of Brain Aging
Inspired by the original "Hallmarks of Aging", researchers have attempted to characterize the aging process more specifically in the nervous tissue. A study in 2018 named the "Hallmarks of Brain Aging" revealed that aging of the brain, at the cellular and molecular levels, resembles to a large degree the hallmarks of aging at the whole organism level.[24][25]
Some reported differences between aging of the brain and other tissues are telomere attrition and cellular senescence.[24] These hallmarks have not been observed in neurons, and it remains to be established whether they occur in other brain cell types such as glial cells. One hypothesis is that differences might arise from the nature of the brain as a non-proliferative, post-mitotic tissue.
The proposed "Hallmarks of Brain Aging" are ten: mitochondrial dysfunction, accumulation of oxidatively damaged molecules, impaired lysosome and proteasome function, dysregulation of neuronal calcium homeostasis, compromised adaptive cellular stress responses, aberrant neuronal network activity, impaired DNA repair, inflammation, stem cell exhaustion and dysregulated energy metabolism.
Below we discuss the original "Hallmarks of Aging" with a focus on neurodegenerative disease:[2][24][25]
1. Mitochondrial Dysfunction
See the full article on mitochondrial dysfunction.
Neurons are metabolically highly active cells that pose high energy demands. Mitochondria play a key role in energy production, but become impaired in brain aging and neurodegenerative diseases. The production of reactive oxygen species (ROS) is associated with aging and neurodegeneration, though its role in aging is not clear.
Mitophagy is the process that results in the selective degradation of mitochondria. Growing evidence suggests that defects in mitophagy occurring in aging contribute to the neurodegenerative process. Studies have demonstrated that DNA damage in the cell can contribute to mitochondrial dysfunction. Therefore, inducing mitophagy has been suggested as a potential strategy to mitigate brain aging.
2. Impaired DNA Repair
Healthy, young cells are able to clear damaged DNA and rapidly repair it through several mechanisms. However, analysis of brain tissue from old individuals have revealed both increased amounts of damaged DNA as well as decreased expression of DNA repair proteins, suggesting DNA repair mechanisms become increasingly dysfunctional with age.[24] Impairment of DNA repair pathways is also sufficient to cause accelerated aging phenotypes, as seen in patients with diseases such as the Werner syndrome.[26]
Several types of DNA damage are associated with neurodegeneration.[2] Disruptive molecules known as reactive oxygen species (ROS) can induce DNA damage and are associated with aging and neurodegenerative disease. Intracellular accumulation of proteins, nucleic acids, and lipids with signs of oxidative damage are seen in neurons. DNA damage itself can lead to genomic instability and cause an increase in cellular senescence and inflammation, which accelerate brain aging. Additionally, ongoing damage to DNA induces activation of a protein called PARP1 which results in depletion of NAD+, an essential co-factor for sirtuin enzymes with important roles in healthspan.
3. Telomere Attrition
See the full article on Telomeres.
Telomeres are the protective 'caps' on the end of chromosomes and are composed of protein and DNA. Each time a cell divides, the telomeres generally become shorter. The shortening of telomeres occurs as part of biological aging and leads to cellular senescence. It is associated with neurodegeneration and neurodegenerative diseases including Alzheimer's disease and Parkinson's disease.
As discussed previously, telomeres of post-mitotic tissues such as the nervous system do not shorten.[24] However, during neurogenesis, newly generated neutrons after differentiation from neural stem cells are highly sensitive to telomere damage and can become apoptotic.[27]
4. Cellular Senescence
See the full article on Cellular senescence.
Cellular senescence refers to a particular state: cells have stopped dividing and growing in size, become resistant to apoptosis, begin releasing pro-inflammatory factors and express the proteins p21 and p16.[28] Cellular senescence can occur as a result of DNA damage to the cell. With age, the number of senescent neurons increases in the brain.
Similarly to the case of telomere attrition, neurons do not seem to undergo senescence.[24] However, evidence is contradictory as studies in old mice have proposed that up to 40% of cortical, hippocampal and peripheral neurons might be senescent.[2] Cellular senescence has also been linked with exacerbated age-related brain dysfunction. As such, targeting senescent cells is being considered as a therapeutic strategy for patients with neurodegenerative diseases.
5. Stem Cell Exhaustion
Stem cells are required for the creation of new cells in later life. Stem cell function appears to decline over an organism's lifespan and has been linked to several hallmarks such as DNA damage, defective proteostasis and epigenetic deregulation. The loss of stem cells is also associated to age-related neurodegeneration.[2]
New neurons can be generated from neuronal stem cells during adulthood in a process known as neurogenesis.[29] This process is especially important in the hippocampus and the olfactory bulb for maintaining functional learning and memory processes, and it has been shown to decline durning normal aging.[30]
6. Loss of Proteostasis
Proteostasis refers to the balance of protein production and degradation in cells and tissues. A balance of proteins is crucial for the normal functioning of cells. The misfolding, aggregation or deposition of proteins has been shown to be connected to several neurodegenerative disorders.
Impaired cellular “waste disposal” and recycling mechanisms, including autophagy, lysosomal function and the proteasome machinery has been shown to decline in neurons during aging.[24] Intact proteosomal machinery is particularly important for the nervous tissue. For instance, mutations in the proteosomal machinery are sufficient to cause early-onset Parkinson’s disease due to the inability to degrade α-synuclein in the brain.[31]
7. Epigenetic Alterations
Epigenetics describe reversible, heritable changes to the function of genes that do not involve changes to the DNA code itself. These gene modifications include methylation, by which a methyl group is added to a DNA molecule, histone modifications or non-protein-coding-RNAs. Epigenetic changes have been associated with neurodegenerative diseases.[2]
Nutrition is one well-studied epigenetic regulator that can have profound effects in disease and in age-related cognitive decline. Calorie restriction and other environmental factors like exercise or stress management, which generate changes in the epigenome, have been proposed as novel approaches to prevent neurodegenerative diseases.[32]
8. Altered Intercellular Communication
Changes to levels of hormones such as insulin, IGF1 or leptin occur with age and are linked with neurodegeneration. In addition, loss of regulation of the immune system with age is implicated in neurodegenerative diseases. For example, inflammation, a protective response to injury, becomes chronically upregulated with age and high levels of inflammation are related to neurodegenerative diseases such as Alzheimer's disease and Parkinson's disease.[2]
Given the interconnectedness between the brain and immune function, it has been hypothesised that a decline in immune responses in the brain is an important factor in neurodegenerative diseases.[33] A key process of the immune response is inflammation. Chronic inflammation leads to activation of microglia and pro-inflammatory cytokines as well as increased oxidative stress, all earmarks associated to neurodegenerative diseases.[34]
9. Dysregulated Energy Metabolism
See the full article on Metabolic flexibility.
Several nutrient sensing biochemical pathways are linked to aging and longevity. These include insulin-like growth factor 1 (IGF1), mechanistic target of rapamycin (mTOR), AMP activated protein kinase (AMPK) and the sirtuin enzymes. Studies in mice, worm and fly species have demonstrated that modulating these pathways can extend lifespan. Metabolic dysfunction in these pathways is commonly associated with neurological, age-related diseases.[2]
Neurons are continuously subject to high bioenergetic demands. The brain is, in fact, the most energy-consuming organ and is responsible for 20% of the total energy use of an organism at rest, despite only accounting for 2% of the human bodyweight. During aging, neural networks are highly susceptible to perturbation due to dysregulated energy metabolism and can become pathological.[24] For instance, excitatory and inhibitory neural network imbalances can result during aging as a result of aging-related impaired GABAergic signalling.[35]
Interconnectedness of the hallmarks
The hallmarks of aging are highly interconnected and occur in a complex process that is part of neurodegeneration. For example, the loss of proteostasis is strongly linked to inflammation and senescence. The metabolic dysfunction that occurs with age and is present in neurodegenerative diseases is associated with mitochondrial dysfunction and oxidative stress. The reduction in number of stem cells is linked to almost all other hallmarks, such as genomic instability, epigenetic alterations, mitochondrial dysfunction, cellular senescence and telomere attrition.[2]
Aging and Alzheimer's disease
New treatments for neurodegenerative diseases
Current efforts to develop evidence-based treatments for neurodegenerative diseases are ongoing, but no highly effective treatment approaches have been discovered. Given the complexity of age-related neurodegenerative diseases, the current approach of targeting single pathways may be inadequate. Instead, broader therapeutic approaches that target the brain aging process directly may be considered as a new approach to treating neurodegenerative diseases. Further research into understanding nine hallmarks of aging and targeting these processes may be required to create effective new therapies for neurodegeneration. Some approaches being explored are described below:
Supplementing NAD+
NAD+ is an essential molecule involved in energy metabolism in the body. A sufficient supply of NAD+ is necessary for maintenance of mitochondrial health, DNA repair, energy homeostasis and brain health. Levels of NAD+ decline with age in humans[36], but supplementation can increase levels of NAD+. Supplementation with molecules that turn into NAD+, known as NAD+ precursors, include nicotinamide riboside (NR) and nicotinamide mononucleotide (NMN). These supplements may help to extend healthy lifespan and slow brain aging, thereby delaying or protecting against neurodegeneration. Supplementation of NAD+ in different models of Alzheimer's Disease has been shown to ameliorate amyloid-β and tau pathology, in addition to improvement in cognition.[37] Studies in mice have shown that NMN can reduce plaque accumulation and improve cognition, as well as learning and memory.[37] Clinical trials evaluating the potential benefits of NAD+ in humans are currently underway.
Induction of Autophagy
Autophagy is a fundamental highly conserved cellular process that degrades cellular components through the use of lysosomes.[38] This process has been highly connected to the process of aging and age-related diseases, and several compounds that can induce autophagy, such as rapamycin, spermidine, urolithin A, and others, have been shown to positively impact lifespan.[38] Dysfunctional acidification of the autolysosome is present in mouse models of Alzheimer's Disease.[39] Stimulation of autophagy through rapamycin and trehalose for instance, can ameliorate tau pathology in mice.[40][41]
Mitophagy, the selective autophagy degradation of mitochondria, has been shown to be reduced by 30-50% in the hippocampus of Alzheimer's patients.[37] The induction of mitophagy can ameliorate Aβ pathology and cognitive decline in AD mice, in addition to promote the phagocytic activity of microglia and reducing neuroinflammation.[37]
Inhibition of cellular senescence
Senescence of the astrocytes and microglial cells - cells that support neurons in the brain - accumulate in normal brain aging and patients with Parkinson's disease and Alzheimer's disease. The elimination of these cells may represent a new strategy for extending the healthspan of the brain and treating neurodegenerative diseases. Strategies using have shown beneficial effects in animal models:
- Rapamycin has been shown to decelerate cellular senescence as one of perhaps several neuroprotective effects
- Metformin has been shown to suppress cellular senescence via activation of microRNA-processing proteins that reduce plaque build-up in Alzheimer's disease and Parkinson's disease.
Hallmark of aging targeted | Drug(s) | Mechanism of action | Disease | Actual or Estimated trial completion date | ClinicalTrials.gov Link |
---|---|---|---|---|---|
Altered intercellular communication | Niacin | Improving mitochondrial function | Parkinson's disease | April 2020 | https://clinicaltrials.gov/ct2/show/NCT03462680 |
Deregulated nutrient sensing | Resveratrol | Reducing insulin resistance | Mild cognitive impairment | June 2022 | https://clinicaltrials.gov/ct2/show/NCT02502253 |
Mitochondrial dysfunction | Nicotinamide (vitamin B3) | Improving mitochondrial function | Alzheimer's disease or MCI | July 2022 | https://clinicaltrials.gov/ct2/show/NCT03061474 |
Mitochondrial dysfunction | Nicotinamide (vitamin B3) | Improving mitochondrial function | Parkinson's disease | March 2024 | https://clinicaltrials.gov/ct2/show/NCT03568968 |
Cellular Senescence | Dasatinib plus Quercetin | Removing senescent cells | Alzheimer's disease | August 2023 | https://clinicaltrials.gov/ct2/show/NCT04063124 |
Cellular Senescence | Dasatinib plus Quercetin | Removing senescent cells | Mild cognitive impairment | July 2031 | https://clinicaltrials.gov/ct2/show/NCT04685590 |
Cellular Senescence | Dasatinib plus Quercetin | Removing senescent cells | Mild cognitive impairment | June 2023 | https://clinicaltrials.gov/ct2/show/NCT04785300 |
Altered intercellular communication | Nicotinamide (vitamin B3) | Improving mitochondrial function | Glaucoma | December 2026 | https://clinicaltrials.gov/ct2/show/NCT05275738 |
Multiple hallmarks | Rapamycin | Inhibiting mTOR pathway | Mild cognitive impairment or early Alzheimer's disease | December 2022 | https://clinicaltrials.gov/ct2/show/NCT04200911 |
Multiple hallmarks | Rapamycin | Inhibiting mTOR pathway | Mild cognitive impairment or early Alzheimer's disease | August 2024 | https://clinicaltrials.gov/ct2/show/NCT04629495 |
References
- ↑ Mehta, P., Kaye, W., Raymond, J., Wu, R., Larson, T., Punjani, R., Heller, D., Cohen, J., Peters, T., Muravov, O., & Horton, K. (2018). Prevalence of Amyotrophic Lateral Sclerosis - United States, 2014. MMWR. Morbidity and mortality weekly report, 67(7), 216–218. https://doi.org/10.15585/mmwr.mm6707a3
- ↑ 2.00 2.01 2.02 2.03 2.04 2.05 2.06 2.07 2.08 2.09 2.10 2.11 Hou, Y., Dan, X., Babbar, M., Wei, Y., Hasselbalch, S. G., Croteau, D. L., & Bohr, V. A. (2019). Ageing as a risk factor for neurodegenerative disease. Nature Reviews Neurology, 15(10), 565-581. https://www.nature.com/articles/s41582-019-0244-7
- ↑ Mayne, K., White, J., McMurran, C., Rivera, F., & de la Fuente, A. (2020). Aging and Neurodegenerative Disease: Is the Adaptive Immune System a Friend or Foe?. Frontiers In Aging Neuroscience, 12. doi: 10.3389/fnagi.2020.572090
- ↑ 4.0 4.1 Matt Kaeberlein, PhD, Time for a New Strategy in the War on Alzheimer’s Disease, Public Policy & Aging Report, Volume 29, Issue 4, 2019, Pages 119–122, https://doi.org/10.1093/ppar/prz020
- ↑ Qiu, C., Kivipelto, M., & von Strauss, E. (2009). Epidemiology of Alzheimer's disease: occurrence, determinants, and strategies toward intervention. Dialogues in clinical neuroscience, 11(2), 111.
- ↑ Manzoni, C., Lewis, P., & Ferrari, R. (2020). Network Analysis for Complex Neurodegenerative Diseases. Current Genetic Medicine Reports, 8(1), 17-25. doi: 10.1007/s40142-020-00181-z
- ↑ Raimundo, R., Jesus, R., & Veiga, A. (2021). Familial Alzheimer’s Disease due to Presenilin 1 Mutation at the Age of 33: a Case Report. SN Comprehensive Clinical Medicine, 3(10), 2021-2023. doi: 10.1007/s42399-021-00982-5
- ↑ Gan, L., Cookson, M.R., Petrucelli, L. et al. Converging pathways in neurodegeneration, from genetics to mechanisms. Nat Neurosci 21, 1300–1309 (2018). https://doi.org/10.1038/s41593-018-0237-7
- ↑ Moskvina V, Harold D, Russo G, et al. Analysis of Genome-Wide Association Studies of Alzheimer Disease and of Parkinson Disease to Determine If These 2 Diseases Share a Common Genetic Risk. JAMA Neurol. 2013;70(10):1268–1276. doi:10.1001/jamaneurol.2013.448
- ↑ Yamazaki, Y., Zhao, N., Caulfield, T.R. et al. Apolipoprotein E and Alzheimer disease: pathobiology and targeting strategies. Nat Rev Neurol 15, 501–518 (2019). https://doi.org/10.1038/s41582-019-0228-7
- ↑ 11.0 11.1 11.2 Maryam Vasefi, Ehsan Ghaboolian-Zare, Hamzah Abedelwahab, Anthony Osu, Environmental toxins and Alzheimer's disease progression, Neurochemistry International, Volume 141, 2020, ISSN 0197-0186, https://doi.org/10.1016/j.neuint.2020.104852.
- ↑ Kamel, F., & Hoppin, J. A. (2004). Association of pesticide exposure with neurologic dysfunction and disease. Environmental health perspectives, 112(9), 950–958. https://doi.org/10.1289/ehp.7135
- ↑ Plamena R. Angelova, Sources and triggers of oxidative damage in neurodegeneration, Free Radical Biology and Medicine, Volume 173, 2021, Pages 52-63, ISSN 0891-5849, https://doi.org/10.1016/j.freeradbiomed.2021.07.003.
- ↑ Chin-Chan, M., Navarro-Yepes, J., & Quintanilla-Vega, B. (2015). Environmental pollutants as risk factors for neurodegenerative disorders: Alzheimer and Parkinson diseases. Frontiers In Cellular Neuroscience, 9. doi: 10.3389/fncel.2015.00124
- ↑ https://www.miskawaanhealth.com/heavy-metal-contamination/
- ↑ Spencer, P., & Palmer, V. (2021). Direct and Indirect Neurotoxic Potential of Metal/Metalloids in Plants and Fungi Used for Food, Dietary Supplements, and Herbal Medicine. Toxics, 9(3), 57. doi: 10.3390/toxics9030057
- ↑ Schaefer, Zoffer, Yrastorza, Pearlman, Bossart, Stoessel, & Reif. (2019). Mercury Exposure, Fish Consumption, and Perceived Risk among Pregnant Women in Coastal Florida. International Journal Of Environmental Research And Public Health, 16(24), 4903. doi: 10.3390/ijerph16244903
- ↑ Li Y, Li Y, Li X, Zhang S, Zhao J, Zhu X, et al. (2017) Head Injury as a Risk Factor for Dementia and Alzheimer’s Disease: A Systematic Review and Meta-Analysis of 32 Observational Studies. PLoS ONE 12(1): e0169650. https://doi.org/10.1371/journal.pone.0169650
- ↑ Graham NS, Sharp DJ, Understanding neurodegeneration after traumatic brain injury: from mechanisms to clinical trials in dementia. Journal of Neurology, Neurosurgery & Psychiatry 2019;90:1221-1233.
- ↑ Wilson RS, Scherr PA, Hoganson G, Bienias JL, Evans DA, Bennett DA. Early life socioeconomic status and late life risk of Alzheimer's disease. Neuroepidemiology. 2005;25(1):8-14. doi: 10.1159/000085307.
- ↑ Stern, Yaakov, Cognitive reserve in ageing and Alzheimer's disease, The Lancet Neurology, Volume 11, Issue 11, 1006 - 1012, https://doi.org/10.1016/S1474-4422(12)70191-6
- ↑ Mehta, K.M., Stewart, A.L., Langa, K.M., Yaffe, K., Moody-Ayers, S., Williams, B.A. and Covinsky, K.E. (2009), “Below average” self-assessed school performance and Alzheimer's disease in the Aging, Demographics, and Memory Study. Alzheimer's & Dementia, 5: 380-387. https://doi.org/10.1016/j.jalz.2009.07.039
- ↑ Staff, R., Murray, A., Deary, I., & Whalley, L. (2004). What provides cerebral reserve?. Brain, 127(5), 1191-1199. doi: 10.1093/brain/awh144
- ↑ 24.0 24.1 24.2 24.3 24.4 24.5 24.6 24.7 24.8 Mattson, M., & Arumugam, T. (2018). Hallmarks of Brain Aging: Adaptive and Pathological Modification by Metabolic States. Cell Metabolism, 27(6), 1176-1199. doi: 10.1016/j.cmet.2018.05.011
- ↑ 25.0 25.1 25.2 López-Otín, C., Blasco, M., Partridge, L., Serrano, M., & Kroemer, G. (2013). The Hallmarks of Aging. Cell, 153(6), 1194-1217. doi: 10.1016/j.cell.2013.05.039
- ↑ Scheibye-Knudsen M. Neurodegeneration in accelerated aging. Dan Med J. 2016;63:1–10.
- ↑ Cheng A, Shinya K, Wan R, Tang SC, Miura T, Tang H, Khatri R, Gleichman M, Ouyang X, Liu D, et al. Telomere protection mechanisms change during neurogenesis and neuronal maturation: newly generated neurons are hypersensitive to telomere and DNA damage. J Neurosci. 2007;27:3722–3733.
- ↑ Childs BG, Baker DJ, Kirkland JL, Campisi J, van Deursen JM. Senescence and apoptosis: dueling or complementary cell fates? EMBO Rep. 2014;15:1139–1153.
- ↑ Ming GL, Song H. Adult neurogenesis in the mammalian brain: significant answers and significant questions. Neuron. 2011;70:687–702.
- ↑ Lazarov O, Mattson MP, Peterson DA, Pimplikar SW, van Praag H. When neurogenesis encounters aging and disease. Trends Neurosci. 2010;33:569–579.
- ↑ Shimura H, Schlossmacher MG, Hattori N, Frosch MP, Trockenbacher A, Schneider R, Mizuno Y, Kosik KS, Selkoe DJ. Ubiquitination of a new form of alpha-synuclein by parkin from human brain: implications for Parkinson’s disease. Science. 2001;293:263–269.
- ↑ Dauncey MJ. Nutrition, the brain and cognitive decline: insights from epigenetics. Eur J Clin Nutr. 2014; 68:1179–1185.
- ↑ Amor, S. & Woodroofe, M. N. Innate and adaptive immune responses in neurodegeneration and repair. Immunology. 2014; 141, 287–291.
- ↑ Currais, A. Ageing and inflammation – a central role for mitochondria in brain health and disease. Ageing Res. Rev. 2025; 21, 30–42.
- ↑ Heise KF, Zimerman M, Hoppe J, Gerloff C, Wegscheider K, Hummel FC. The aging motor system as a model for plastic changes of GABA-mediated intracortical inhibition and their behavioral relevance. J Neurosci. 2013;33:9039–9049.
- ↑ Camacho-Pereira J, Tarragó MG, Chini CCS, Nin V, Escande C, Warner GM, Puranik AS, Schoon RA, Reid JM, Galina A, Chini EN. CD38 Dictates Age-Related NAD Decline and Mitochondrial Dysfunction through an SIRT3-Dependent Mechanism. Cell Metab. 2016 Jun 14;23(6):1127-1139. doi: 10.1016/j.cmet.2016.05.006. PMID: 27304511; PMCID: PMC4911708.
- ↑ 37.0 37.1 37.2 37.3 Fang EF, Hou Y, Palikaras K, Adriaanse BA, Kerr JS, Yang B, Lautrup S, Hasan-Olive MM, Caponio D, Dan X, Rocktäschel P, Croteau DL, Akbari M, Greig NH, Fladby T, Nilsen H, Cader MZ, Mattson MP, Tavernarakis N, Bohr VA. Mitophagy inhibits amyloid-β and tau pathology and reverses cognitive deficits in models of Alzheimer's disease. Nat Neurosci. 2019 Mar;22(3):401-412. doi: 10.1038/s41593-018-0332-9. Epub 2019 Feb 11. PMID: 30742114; PMCID: PMC6693625.
- ↑ 38.0 38.1 Aman Y, Schmauck-Medina T, Hansen M, Morimoto RI, Simon AK, Bjedov I, Palikaras K, Simonsen A, Johansen T, Tavernarakis N, Rubinsztein DC, Partridge L, Kroemer G, Labbadia J, Fang EF. Autophagy in healthy aging and disease. Nat Aging. 2021 Aug;1(8):634-650. doi: 10.1038/s43587-021-00098-4. Epub 2021 Aug 12. PMID: 34901876; PMCID: PMC8659158.
- ↑ Lee JH, Yang DS, Goulbourne CN, Im E, Stavrides P, Pensalfini A, Chan H, Bouchet-Marquis C, Bleiwas C, Berg MJ, Huo C, Peddy J, Pawlik M, Levy E, Rao M, Staufenbiel M, Nixon RA. Faulty autolysosome acidification in Alzheimer's disease mouse models induces autophagic build-up of Aβ in neurons, yielding senile plaques. Nat Neurosci. 2022 Jun;25(6):688-701. doi: 10.1038/s41593-022-01084-8. Epub 2022 Jun 2. PMID: 35654956; PMCID: PMC9174056.
- ↑ Ozcelik S, Fraser G, Castets P, Schaeffer V, Skachokova Z, Breu K, Clavaguera F, Sinnreich M, Kappos L, Goedert M, Tolnay M, Winkler DT. Rapamycin attenuates the progression of tau pathology in P301S tau transgenic mice. PLoS One. 2013 May 7;8(5):e62459. doi: 10.1371/journal.pone.0062459. PMID: 23667480; PMCID: PMC3646815.
- ↑ Schaeffer V, Lavenir I, Ozcelik S, Tolnay M, Winkler DT, Goedert M. Stimulation of autophagy reduces neurodegeneration in a mouse model of human tauopathy. Brain. 2012 Jul;135(Pt 7):2169-77. doi: 10.1093/brain/aws143. Epub 2012 Jun 10. PMID: 22689910; PMCID: PMC3381726.